GNSS corrections are essential for achieving high-precision positioning. Uncorrected GNSS (Global Navigation Satellite System) signals typically provide an accuracy of 5-10 meters which is insufficient for applications like agriculture, surveying, autonomous navigation, and more. By computing error corrections on these GNSS signals, accuracy can be improved by up to 100x, achieving centimeter-level precision.
This blog delves into the primary GNSS correction methods—Differential GNSS (DGNSS), Satellite-Based Augmentation Services (SBAS), Real-Time Kinematic (RTK), Precise Point Positioning (PPP), and PPP-RTK—examining their features, advantages, limitations, and use cases. We’ll discuss how these methods balance accuracy, coverage, complexity, and cost, making each suitable for a range of applications. Finally, we’ll explore how to choose the best GNSS correction method for your needs and highlight the role of cutting-edge GNSS correction services like Skylark™ Precise Positioning Service.
But first, how does standard GNSS positioning work?
For an in-depth explanation of GNSS positioning, read this blog post. Here’s an abbreviated overview:
Raw, uncorrected GNSS signals from each satellite contain a unique pseudorandom noise (PRN) code which communicates three key pieces of information: the satellite’s ID (who), its position in orbit (where), and the onboard time the signal was transmitted (when). A GNSS receiver then calculates the travel time of a signal from a satellite by comparing its internally generated PRN code with the same code embedded in the satellite’s signal. To achieve synchronization, the receiver shifts its code along the sequence until it aligns with the satellite’s PRN code. The amount of this shift corresponds to the time passed since the signal was transmitted, i.e., its travel time. This is called code-phase positioning. Using this information, the receiver can calculate the distance travelled by the signal, known as a pseudorange. Once the distances to four or more satellites are determined, the receiver can infer its own position in three dimensions.
However, GNSS systems are subject to various errors that degrade positioning accuracy, with atmospheric interference being the most significant. As GNSS signals pass through the ionosphere (outer layer) and troposphere (layer nearest the Earth’s surface), they are slowed down and refracted, introducing inaccuracies. Even the highly precise atomic clocks onboard satellites are prone to slight deviations that impact position calculations, as are the satellites’ orbits, which can deviate marginally from their predicted paths. Lastly, in complex environments, GNSS receivers receive multiple copies of the same signal as it bounces off nearby objects such as buildings and trees, affecting its location calculation. These errors compound to an overall positioning error that ranges between 5 to 10 meters. GNSS correction methods are used to reduce these errors.
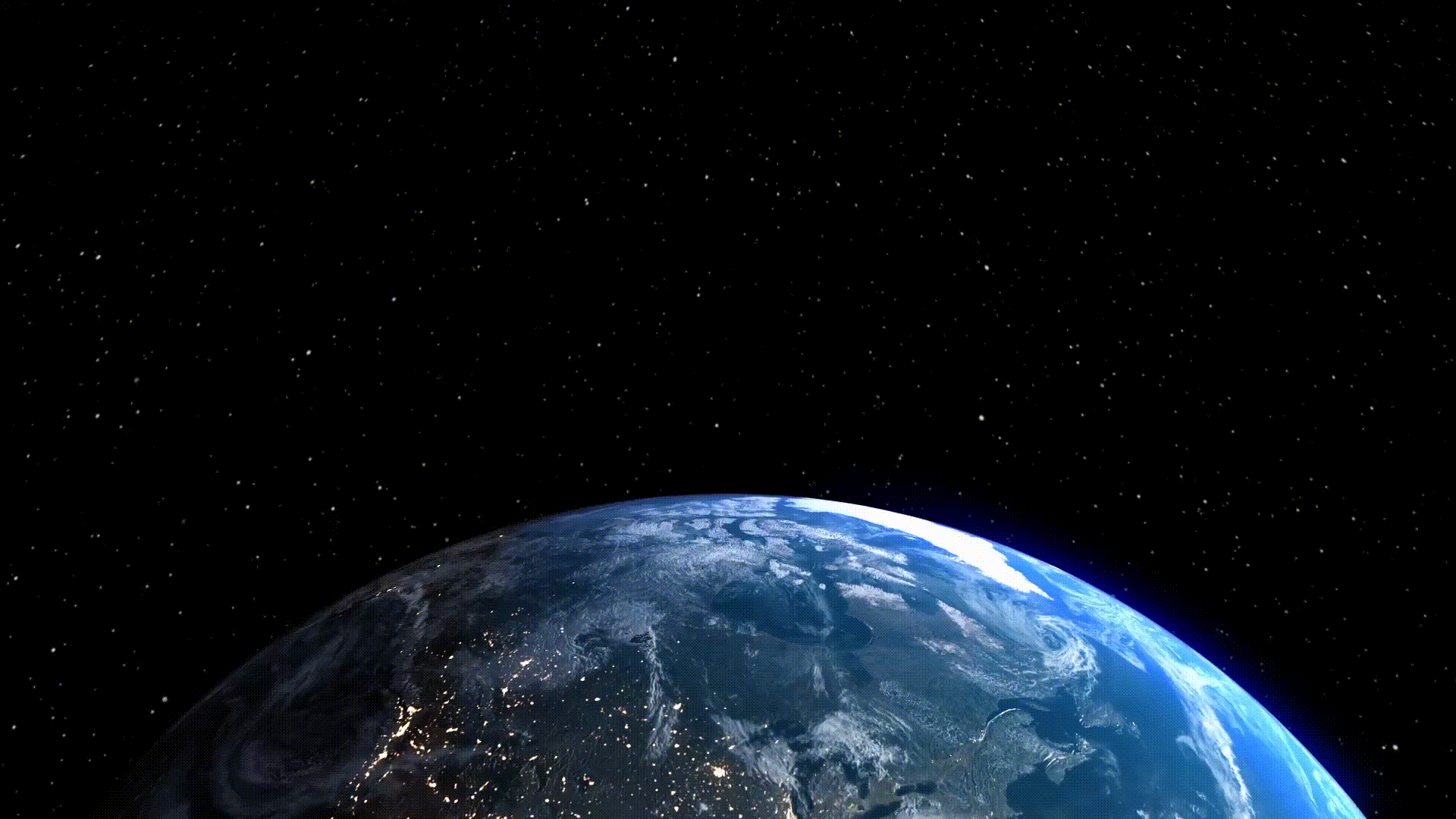
Differential GNSS (DGNSS)
DGNSS provides corrections based on the concept of relative positioning by comparing the GNSS data from a user receiver, or rover, with that of a reference station, located at a precisely known position. The reference station calculates pseudoranges to the satellites it is tracking using code-phase measurements, just as explained above. By comparing these calculated pseudoranges to the true distances (known from its surveyed position), the reference station determines the error for each satellite in view. These errors are broadcast as pseudorange corrections for each satellite (e.g., +2.1 meters for satellite A, −1.5 meters for satellite B, etc.).
The rover in the field calculates its own pseudoranges to the same satellites using code-phase measurements. For each satellite it is tracking, the rover applies the correction from the reference station to its pseudorange calculation, effectively removing most shared errors such as satellite clock errors, orbit errors, and atmospheric delays. By applying these corrections, the rover significantly reduces measurement errors, improving GNSS accuracy to under a meter.
Pros: Simple to implement, widely available, sufficient for non-critical applications, low processing power required at receiver, fast convergence time, ideal for receivers with a constrained antenna such as mobile devices.
Cons: Lower accuracy than RTK or PPP, limited range from the base station.
Use Cases: Marine navigation, some agricultural operations, and recreational positioning.
Asset Tracking with Differential GNSS Corrections
DGNSS is a reliable solution for asset tracking, enabling real-time monitoring of vehicles, equipment, and cargo in industries such as logistics and construction. By providing sub-meter accuracy, DGNSS ensures efficient operations and reduces the risk of loss or theft. For example, construction companies can track machinery across large sites, while logistics providers monitor delivery vehicles and cargo containers. Additionally, DGNSS is light on power consumption, making it easy to implement in small battery-operated trackers. Its affordability and ease of implementation make DGNSS a practical choice for asset tracking applications that do not demand the centimeter-level precision of advanced correction methods like RTK or PPP.
Satellite-Based Augmentation System (SBAS)
Similar to DGNSS, SBAS improves GNSS accuracy by leveraging a network of ground-based reference stations to monitor satellite signals for errors. Corrections are computed at a central facility and then broadcast to SBAS-enabled receivers via geostationary satellites, facilitating wide-area coverage without the need for direct communication with local reference stations.
Receiving SBAS corrections requires line of sight with geostationary satellites, which may be low above the horizon in northern or southern latitudes. This can limit the effectiveness of SBAS in environments where obstacles such as buildings, trees, or rugged terrain obstruct the signal.
SBAS corrections are generally more coarse than DGNSS corrections, as they are designed to apply over large geographic regions rather than being tailored to localized environments. This trade-off between precision and coverage makes SBAS well-suited for applications requiring reliable accuracy across wide regions, without the need for sub-meter level precision.
SBAS services are available globally, with regional implementations such as WAAS (Wide Area Augmentation System) in the U.S., EGNOS (European Geostationary Navigation Overlay Service) in Europe, and MSAS (Multi-functional Satellite Augmentation System) in Japan. These systems are particularly useful for applications requiring enhanced accuracy over large areas without the need for additional hardware.
Pros: Free-to-use (in most regions), no additional infrastructure needed for users, wide-area coverage.
Cons: Limited accuracy compared to RTK or PPP, dependent on regional SBAS infrastructure.
Use Cases: Aviation, maritime navigation, and agriculture applications that do not require centimeter-level precision.
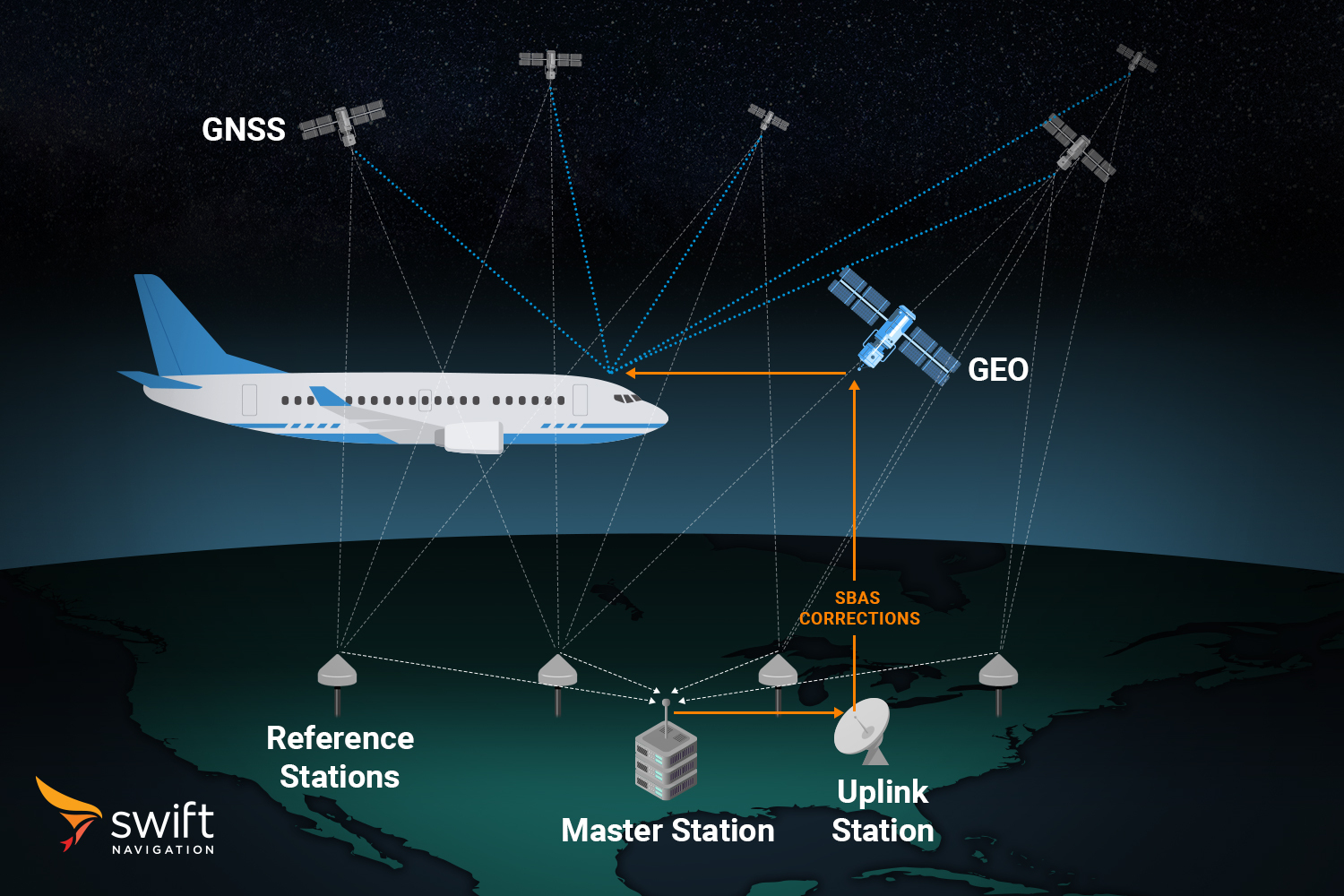
SBAS in Aviation
SBAS is a cornerstone of modern aviation safety, providing not only precision but also integrity, ensuring that users are immediately alerted if GNSS data is unreliable. This is critical for supporting precision approaches and landings, providing reliable vertical and horizontal guidance for aircraft. For instance, WAAS-enabled GNSS receivers are mandatory for many flight operations in North America, ensuring that pilots can navigate and land even in poor visibility conditions.
Real-Time Kinematic (RTK)
RTK corrects for GNSS errors by using a single, or network of, fixed reference stations that communicate with GNSS receivers in real-time. It uses a combination of code-phase and carrier-phase measurements. This method provides centimeter-level accuracy with extremely low latency, making it ideal for applications where real-time, high-precision data is crucial.
RTK-grade GNSS receivers first utilize the pseudo-random code for initial positioning. They then transition to more precise measurements based on the carrier signal, which operates at a much higher frequency. While the PRN code has a bit rate of approximately 1 MHz (resulting in a wavelength of about 300 meters), the carrier frequency cycles at over 1.57 GHz for GPS L1, corresponding to a much shorter wavelength of about 19 centimeters. This higher frequency makes carrier-phase measurement inherently more precise than the code-phase.
RTK calculates the exact number of carrier cycles between the satellite and the receiver. However, the challenge lies in resolving the integer ambiguity–the unknown number of full wavelengths (N) between the satellite and receiver–because each carrier cycle is identical. In contrast, the PRN code is intentionally designed to be complex, allowing easier identification of specific cycles.
RTK resolves the integer ambiguity through a process called double differencing. This process compares measurements between the rover, reference station, and multiple satellites to eliminate most clock errors. RTK algorithms then test combinations of N values across all satellites in view, using methods like the Lambda algorithm to find the set that best fits the data. Once resolved, the ambiguities are fixed into the solution, enabling centimeter-level accuracy with the help of real-time corrections.
Pros: Fast convergence, high accuracy (2 cm).
Cons: Limited coverage area (usually within 20-30 km of a base station), requires reliable network connectivity (if using NTRIP).
Use Cases: Autonomous vehicles, outdoor robotics, precision construction or land surveying in controlled areas.
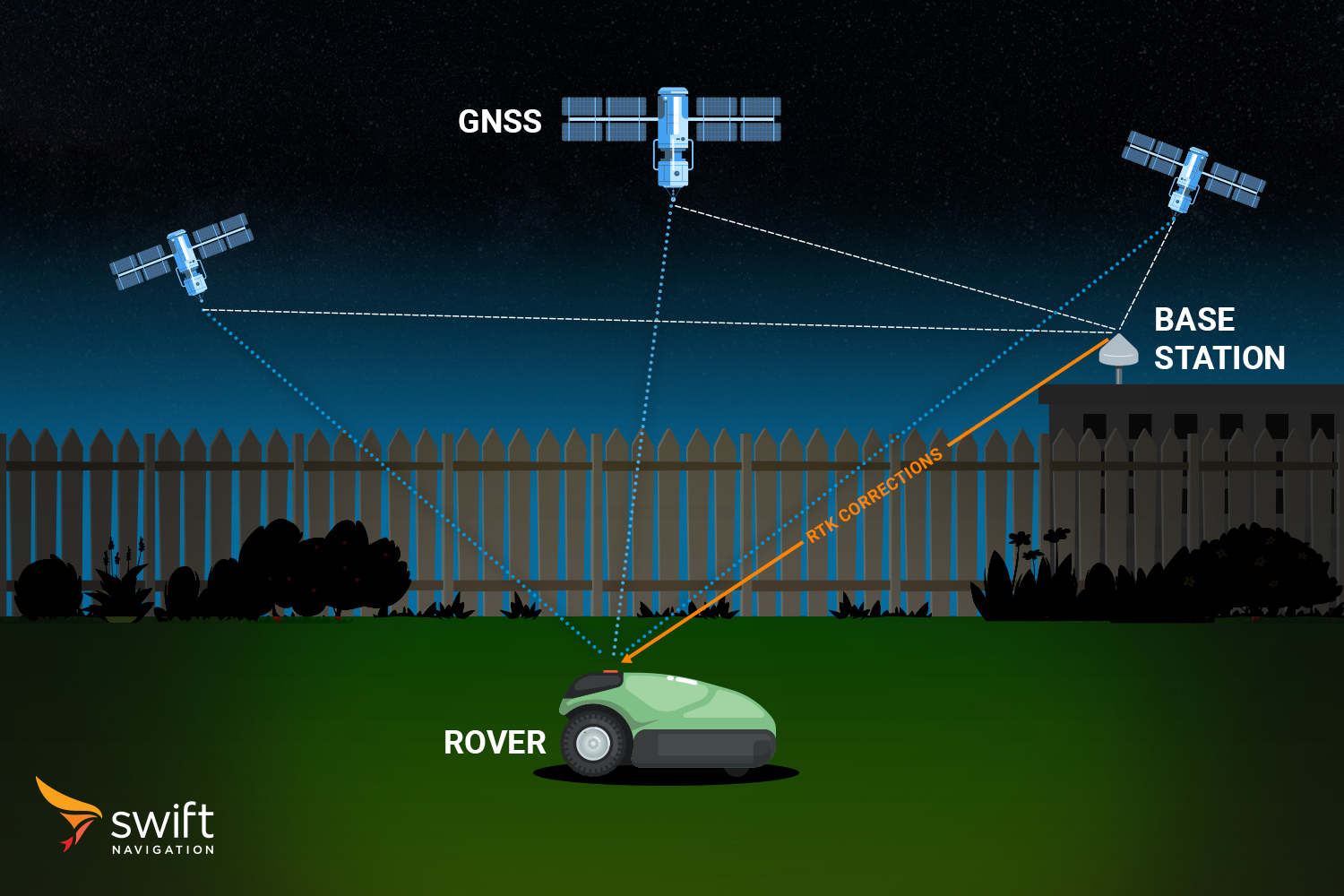
RTK Corrections in Robotics
RTK GNSS corrections are critical in the robotics field, enabling autonomous machines to operate with extraordinary precision. Agricultural robots use RTK for row-by-row crop management, while drones equipped with RTK navigate urban corridors and conduct detailed infrastructure inspections. In construction, RTK-powered robots lay foundations and position machinery with centimeter-level accuracy, enhancing efficiency and reducing errors.
Precise Point Positioning (PPP)
PPP enhances GNSS positioning by applying corrections to the satellite orbit, clock, and atmospheric errors. Unlike DGNSS or RTK, which rely on real-time corrections from nearby base stations, PPP leverages a network of reference stations sparsely distributed around the world. These stations monitor GNSS satellites to generate precise orbit and clock data, which is then transmitted to users via satellite or the internet.
PPP achieves high accuracy by combining code-phase and carrier-phase measurements, along with sophisticated error modeling. It provides accuracy levels ranging from decimeters to a few centimeters, with global coverage. However, PPP requires significantly longer convergence times–typically several minutes to half an hour–as the receiver must resolve carrier-phase ambiguities without the assistance from a local base station as in RTK. Instead, PPP receivers independently resolve the integer ambiguity by continuously collecting and analyzing carrier-phase observations over a long period of time. By tracking multiple satellites and applying the precise orbit and clock corrections received, the receiver iteratively refines its position, gradually achieving centimeter-level accuracy.
Pros: Global coverage, high accuracy (centimeter-level), no need for local base stations.
Cons: Long convergence times (up to 30 minutes), lower real-time capability compared to RTK.
Use Cases: Offshore positioning, global surveying, scientific research, and geodetic applications.
PPP in Maritime Navigation
PPP is ideal for maritime navigation, providing centimeter-level accuracy globally without relying on local correction infrastructure like RTK. Ships can afford the longer convergence times of PPP, as they often remain stationary or move slowly during port operations or anchoring. This precision is critical for navigating narrow channels, docking, and offshore operations. For instance, offshore platforms and survey vessels rely on PPP for precise positioning during underwater inspections, resource exploration, and construction, ensuring safety and success in remote locations where other correction methods are unavailable.
PPP-RTK
PPP-RTK combines the global reach of PPP with the rapid convergence times and high-precision of RTK. By integrating precise satellite orbit and clock corrections derived from PPP with real-time atmospheric corrections and regional reference network data as RTK, PPP-RTK delivers centimeter-level positioning accuracy with fast convergence times.
The PPP-RTK framework leverages a wide network of reference stations to produce satellite corrections and localized atmospheric delay models, which are transmitted to user receivers via satellite links or the internet. Unlike RTK, which requires a dense network of local base stations, PPP-RTK functions efficiently across expansive regions, making it well-suited for applications requiring seamless national/global operations.
A key component of PPP-RTK’s architecture is State Space Representation (SSR), a method of transmitting corrections by modeling the individual sources of GNSS errors as separate parameters. Unlike Observation Space Representation (OSR), which transmits aggregated corrections tied to the user’s specific location, SSR sends corrections as discrete components that apply to an entire region, or tile. Receivers dynamically combine these components based on their specific location and observed satellites. SSR’s distributed approach supports far more simultaneous users without overloading the network, making it ideal for widespread applications.
Another enhancement to PPP-RTK’s performance is wide-lane ambiguity resolution, which uses wide-lane combination—a linear amalgamation of observations from the same satellite, captured simultaneously on two distinct frequencies (e.g., L1 and L5). This process essentially creates a new composite signal with a longer wavelength than the originals (e.g., around 75 cm for the wide-lane combination of GPS L1 and L5, versus only 19 cm for GPS L1). This longer wavelength simplifies ambiguity resolution, reducing the number of possible integer values and making the process less sensitive to ionospheric disturbances and measurement noise. By resolving wide-lane ambiguities first, the system constrains and accelerates the resolution of standard carrier-phase ambiguities.
This approach improves PPP-RTK’s robustness and accuracy, enabling it to deliver precise and reliable positioning even in challenging environments. Its unique ability to combine global reach, fast convergence, and high precision makes PPP-RTK a powerful solution for diverse applications, from autonomous vehicles to fleet management.
Pros: Shorter convergence times than PPP, high accuracy with broader coverage than RTK with significantly lower number of base stations required.
Cons: Requires more advanced GNSS receivers, slightly lower accuracy than RTK.
Use Cases: Wide-area autonomous navigation, automotive safety, fleet management.
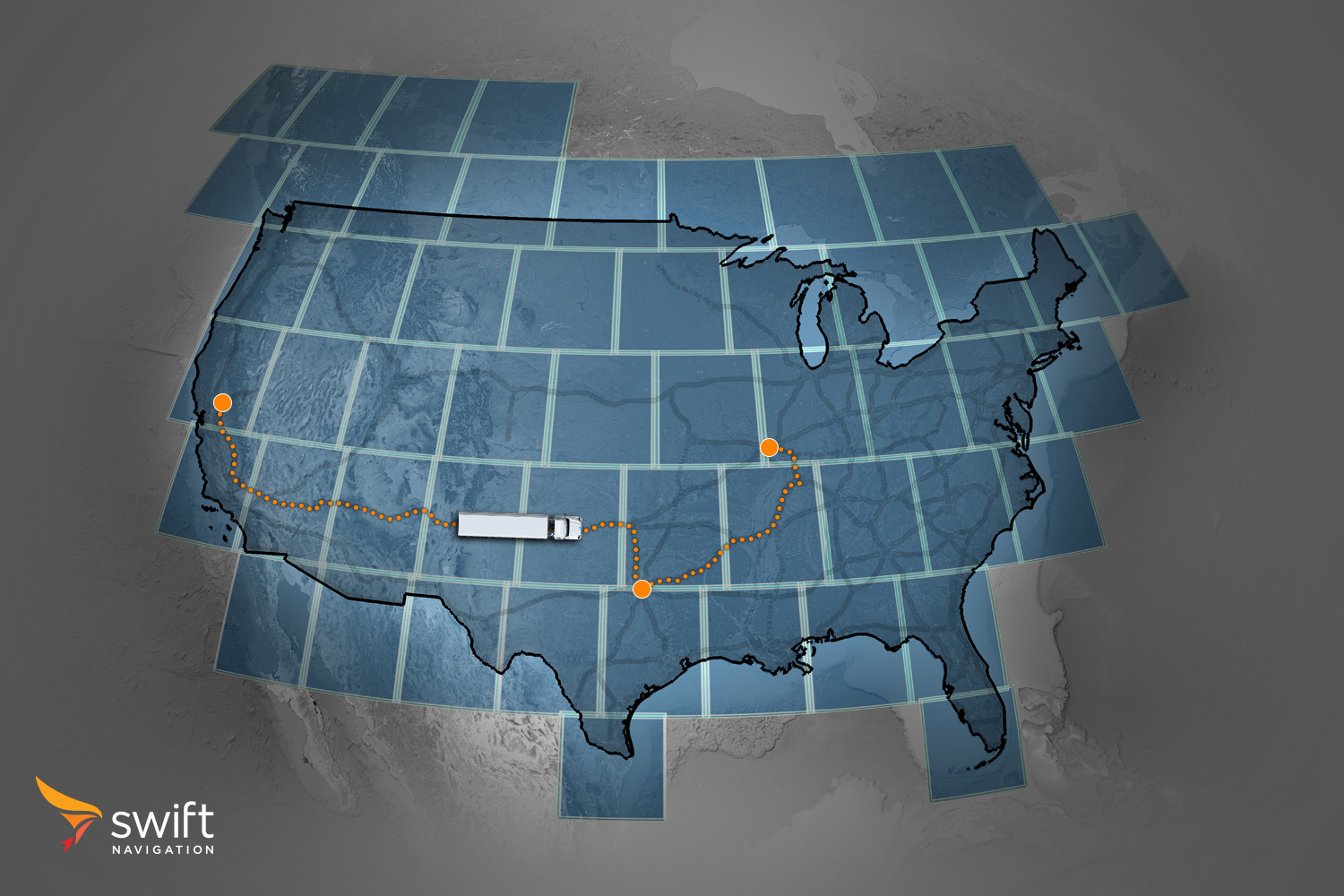
PPP-RTK in Automotive Safety
PPP-RTK is revolutionizing autonomous navigation by providing reliable, high-precision positioning across diverse environments. Its flexibility and wide coverage make it indispensable for advanced driver-assistance systems (ADAS) and autonomous vehicles. For example, self-driving cars rely on PPP-RTK to maintain accurate lane-level positioning in real time, even when navigating through challenging environments like urban canyons or mountain passes.
Summary of GNSS Correction Methods
GNSS Correction Method | Accuracy | Time To First Fix | Baseline | Typical Use Cases |
Standard GNSS | 5-10 m | ~3-10 seconds | Worldwide | Consumer navigation apps, fitness trackers, general positioning |
SBAS | ~1-3 m | ~30 seconds | Continental | Aviation safety, broad-scale agricultural management |
DGNSS | <1 m | ~5-20 seconds | Local / Regional / Continental | Entry-level precision agriculture, basic marine navigation |
PPP | ~10 cm (static) | ~10-30 minutes | Global | Offshore energy operations, global asset tracking, remote surveying |
PPP-RTK | ~3-7 cm | ~10-30 seconds | Continental | Nationwide autonomous fleet navigation, UAV inspections, smart agriculture |
RTK | ~1-2 cm | ~5 seconds | Local / Regional | High-precision agriculture, surveying, robotics, autonomous vehicles |
Choosing the Right GNSS Correction Method
When choosing a GNSS correction service, it’s crucial to consider the specific needs of your application, such as accuracy, coverage, and cost. Swift Navigation’s GNSS correction service comes in three variants suitable for all use cases: Skylark Dx (DGNSS service), Skylark Nx RTK (VRS-based Network RTK service), and Skylark Cx (PPP-RTK service), each designed to meet distinct use cases. Whether you require maximum precision for robotics and surveying, continent-wide scalability for automotive safety, or efficient, low-power solutions for IoT devices, Skylark provides a tailored correction service to fit your needs.
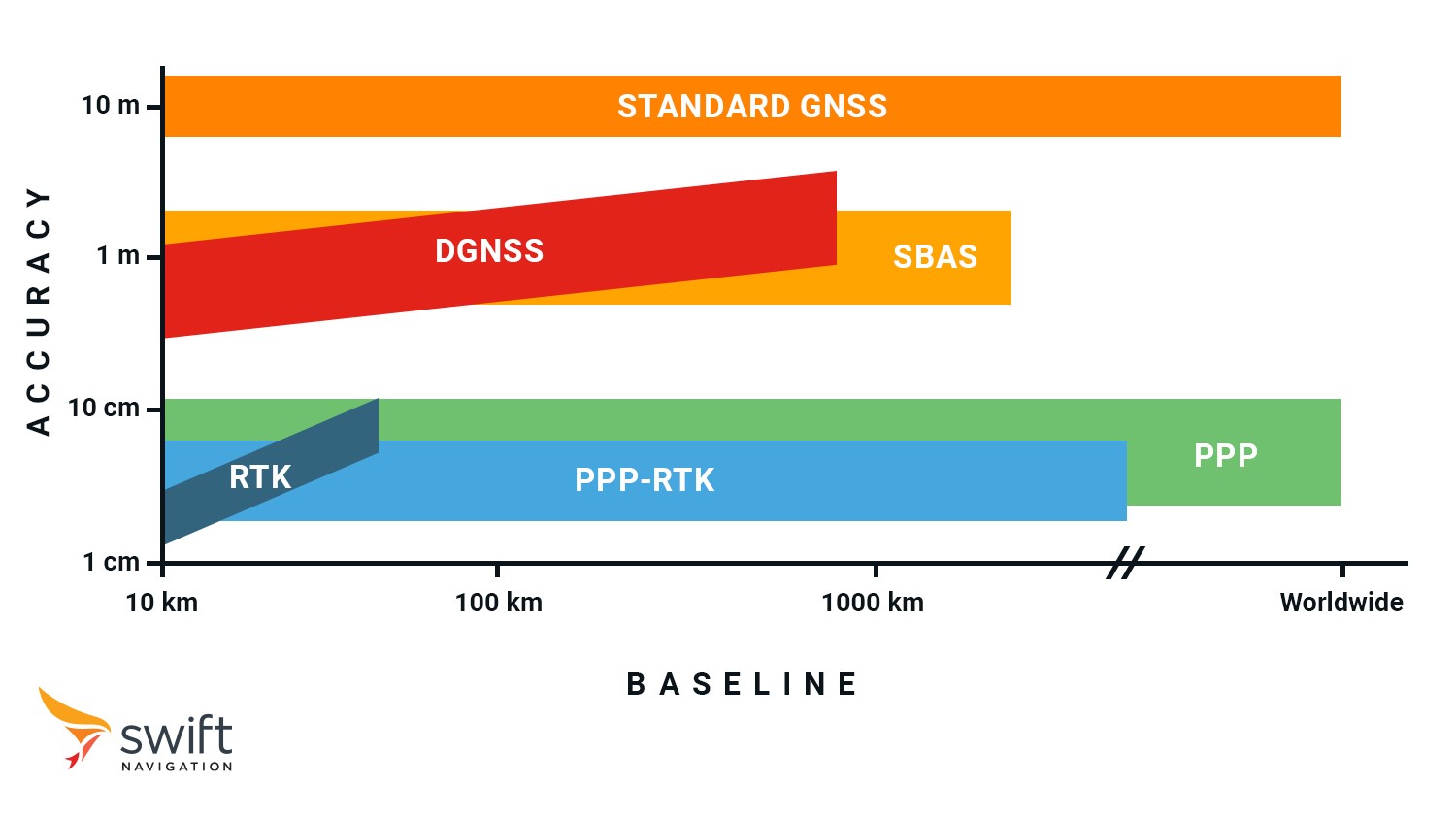
For a deeper dive into Skylark’s variants and guidance on choosing the best GNSS correction service for your application, check out our blog post: Which Skylark Variant Fits Your Use Case?